RAdolphs Neurons Monitor for Mistakes FNL
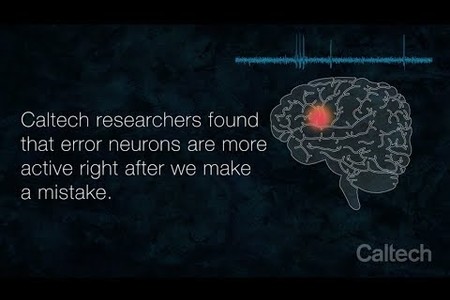
Everyone makes little everyday mistakes out of habit—a waiter says, "Enjoy your meal," and you respond with, "You, too!" before realizing that the person is not, in fact, going to be enjoying your meal. Luckily, there are parts of our brains that monitor our behavior, catching errors and correcting them quickly.
A Caltech-led team of researchers has now identified the individual neurons that may underlie this ability. The work provides rare recordings of individual neurons located deep within the human brain and has implications for psychiatric diseases like obsessive-compulsive disorder.
The work was a collaboration between the laboratories of Ralph Adolphs (PhD '93), Bren Professor of Psychology, Neuroscience, and Biology, and the Allen V. C. Davis and Lenabelle Davis Leadership Chair and director of the Caltech Brain Imaging Center of the Tianqiao and Chrissy Chen Institute for Neuroscience; and Ueli Rutishauser (PhD '08), associate professor of neurosurgery, neurology, and biomedical sciences, and Board of Governors Chair in Neurosciences at Cedars-Sinai Medical Center. A paper describing the research was published online in the journal Neuron on December 4.
"Many people know the feeling of making a mistake and quickly catching oneself—for example, when you are typing and press the wrong key, you can realize you made a mistake without even needing to see the error on the screen," says Rutishauser, who is also a visiting associate in Caltech's Division of Biology and Biological Engineering. "This is an example of how we self-monitor our own split-second mistakes. Now, with this research, we know which neurons are involved in this, and we are starting to learn more about how the activity of these neurons helps us change our behavior to correct errors."
In this work, led by Caltech graduate student Zhongzheng (Brooks) Fu, the researchers aimed to get a precise picture of what happens on the level of individual neurons when a person catches themselves after making an error. To do this, they studied people who have had thin electrodes temporarily implanted into their brains (originally to help localize epileptic seizures). The work was done in collaboration with neurosurgeon Adam Mamelak, professor of neurosurgery at Cedars-Sinai, who has conducted such electrode implantations for clinical monitoring of epilepsy for over a decade and closely collaborated on the research studies.
While neural activity was measured in their medial frontal cortex (MFC), a brain region known to be involved in error monitoring, the epilepsy patients were given a so-called Stroop task to complete. In this task, a word is displayed on a computer screen, and the patients are asked to identify the color of the text. Sometimes, the text and the color are the same (the word "green" for example, is shown in green). In other cases, the word and the color are different ("green" is shown in red text). In the latter case, the correct answer would be "red," but many people make the error of saying "green." These are the errors the researchers studied.
The measurements allowed the team to identify specific neurons in the MFC, called self-monitoring error neurons, that would fire immediately after a person made an error, well before they were given feedback about their answer.
For decades, scientists have studied how people self-detect errors using electrodes placed on the surface of the skull that measure the aggregate electrical activity of thousands of neurons. These so-called electroencephalograms reveal that one particular brainwave signature, called the error-related negativity (ERN), is commonly seen on the skull over the MFC right after a person makes an error. In their experiments, Fu and his colleagues simultaneously measured the ERN as well as the firing of individual error neurons.
They discovered two fundamental new aspects of the ERN. First, an error neuron's activity level was positively correlated with the amplitude of the ERN: the larger the ERN for a particular error, the more active were the error neurons. This finding reveals that an observation of the ERN—a noninvasive measurement—provides information about the level of activity of error neurons found deep within the brain. Second, they found that this ERN–single-neuron correlation, in turn, predicted whether the person would change their behavior—that is, if they would slow down and focus more to avoid making an error on their next answer. If the error neurons fired but the brain-wide ERN signature was not seen or was weak, the person might still recognize that they made an error, but they would not modify their behavior for the next task. This suggests that the error neurons need to communicate their error detection to a large brain network in order to influence behavior.
The researchers found further specific evidence for parts of the circuit involved.
"We found error neurons in two different parts of the MFC: the dorsal anterior cingulate cortex (dACC) and the pre-supplementary motor area (pre-SMA)," says Fu. "The error signal appeared in the pre-SMA 50 milliseconds earlier than in the dACC. But only in the dACC was the correlation between the ERN and error neurons predictive of whether a person would modify their behavior. This reveals a hierarchy of processing—an organizational structure of the circuit at the single-neuron level that is important for executive control of behavior."
The research could also have implications for understanding obsessive-compulsive disorder, a condition in which a person continuously attempts to correct perceived "errors." For example, some individuals with this condition will feel a need to repeatedly check, in a short time period, if they have locked their door. Some people with obsessive-compulsive disorder have been shown to have an abnormally large ERN potential, indicating that their error-monitoring circuitry is overactive. The discovery of error neurons might facilitate new treatments to suppress this overactivity.
The researchers next hope to identify how the information from error neurons flows through the brain in order to produce behavioral changes like slowing down and focusing. "So far, we have identified two brain regions in the frontal cortex that appear to be part of a sequence of processing steps, but, of course, the entire circuit is going to be much more complex than that," says Adolphs. "One important future avenue will be to combine studies that have very fine resolution, such as this one, with studies using fMRI [functional magnetic resonance imaging] that give us a whole-brain field of view."
The paper is titled, "Single-neuron correlates of error monitoring and post-error adjustments in human medial frontal cortex." In addition to Fu, Adolphs, Rutishauser, and Mamelak, other co-authors are Caltech scientist Daw-An Wu; Ian Ross of the Huntington Memorial Hospital in Pasadena; and Jeffrey Chung of Cedars-Sinai Medical Center. Funding was provided by the National Institutes of Health, the National Science Foundation, and the McKnight Endowment Fund for Neuroscience. This study was approved by the institutional review boards of Caltech, Cedars-Sinai Medical Center, and Huntington Memorial Hospital.